Why integrate Biomass Crops into Agroforestry Systems?
Key messages:
- One promising dimension in agroforestry that is yet to realise its full potential is the integration of biomass crops.
- The deliberate incorporation of biomass crops into agroforestry systems can deliver a wide range of environmental benefits including improving soil health, carbon sequestration, enhancing biodiversity, and improving water quality.
- There is the need for policies and funding provisions to support integration of biomass crops in agroforestry systems.
Introduction
The UK government has made a commitment to achieve net-zero greenhouse gas emissions by 2050. As part of a comprehensive strategy addressing the effects of climate change, tree planting is a key component. The UK government has outlined an ambitious 25-year plan to improve the environment. This aims for a 12% tree cover by 2060, which will involve the planting of 180,000 hectares of trees by the end of 2042. In Wales, farmers are expected to allocate at least 10% of their land to tree cover in return for payments from the (currently delayed) Sustainable Farming Scheme (SFS). The Scottish government has similarly set targets to achieve a 21% forest and woodland cover by 2032, with a strong desire for greater integration of land use practices. Northern Ireland’s forest for our future programme aims to plant 9,000 hectares of new woodland (18 million trees) by 2030. To reach these goals, agroforestry can play a key role. To reach these goals, agroforestry will play a key role. Agroforestry is a land management approach that combines trees and shrubs with crop and livestock farming systems.
One promising dimension in agroforestry that is yet to realise its full potential is the integration of biomass crops (such as willow, poplar, alder, and black locust). When incorporated into agroforestry systems, biomass crops contribute in various ways, including, support for pollinator populations, biodiversity enhancement, flood risk reduction, minimized runoff and soil erosion, improved water quality, increased soil carbon sequestration, improved soil health and climate change mitigation. Moreover, they offer biomass for use as timber, wood fuel, bedding, and fodder. Despite these substantial benefits, the integration of biomass crops into agroforestry remains underexplored, with limited available information. This article focuses on the benefits of deliberate integration of biomass crops into agroforestry system.
Understanding Agroforestry Systems
Agroforestry is a deliberate practice that involves integrating woody vegetation, such as trees and shrubs, with agricultural and animal production systems to benefit from the resulting ecological and economic interactions. This can involve integrating livestock and crops into tree-only systems, as well as incorporating trees into farming systems that have crop, livestock, and mixed farms. There are five distinct types of agroforestry,including;
- Silvopastoral agroforestry, which combines trees with livestock.
- Silvoarable agroforestry, the combination of trees and crops.
- Hedgerows, shelterbelts, and riparian buffer strips, which entail growing trees between parcels of land.
- Forest farming, where crops are cultivated within a forest environment.
- Home gardens, characterized by the combination of trees and food production in proximity to households.
The tree component of agroforestry can be from a diverse range of tree and shrub species, including biomass tree crops (both short rotation forestry (SRF) and short rotation coppice (SRC)) integrated into existing landscapes. For instance, in silvoarable agroforestry, one might find combinations of poplar trees with oilseed rape or cherry with willow SRC. Silvopastoral agroforestry reduce heat stress for livestock,enhance their well-being and productivity, and provide fodder. Hedgerows, shelterbelts and riparian buffer strips are the most visible and recognisable forms of agroforestry in the UK. Only 3% of the UK farmed area practises agroforestry.
The establishment of agroforestry systems requires careful planning and well-designed layouts. Depending on the tree type, the agricultural components and their spatial and temporal arrangement, competition for light, nutrients, and water, can intensify, potentially leading to yield reduction. However, with appropriate planning and precise spatial arrangements, the advantages of agroforestry can surpass those of monocropping systems.
The format by which agroforestry is implemented can vary widely, from uniform rows of arable crops and forestry, to expanding the width and amounts of hedgerows on pastureland. The agroforestry handbookprovides practical guidelines and design considerations (Fig.1) for establishing agroforestry systems.
Why integrate biomass crops into agroforestry systems?
Strategic planting of dedicated biomass tree crops in agroforestry systems can increase landscape heterogeneity and enhance ecosystem function. There are several benefits of incorporating biomass crops into agroforestry systems:
Carbon Sequestration and tackling climate change
Incorporating trees or shrubs into farmlands or pastures has proven to be a highly effective strategy for enhancing carbon sequestration compared to monoculture fields of crops or pastures. The integration of biomass crops within agroforestry systems serves as an effective mechanism for capturing and storing carbon from the atmosphere through the process of photosynthesis. These biomass crops play a substantial role in reducing atmospheric carbon dioxide concentrations by accumulating relatively large quantities of carbon in above and below-ground biomass and in the soil. These crops exhibit the potential to sequester significant volumes of carbon dioxide that they absorb during their growth, storing this carbon within the soil. Additionally, the fallen leaves and branches from these biomass crops contribute organic matter to the soil, further enhancing long-term carbon storage.
The root structure of biomass crops continuously expands throughout their life cycle, storing and transferring carbon to the soil. For instance, a study conducted in Southern England illustrated that SRC willow plantations had reduced soil respiration (912 ± 42 g C m-2 yr-1 ) and acted as a net carbon sink of 221 ± 66 g C m-2 yr-1). Another study showed that when biomass crops are established in areas with low carbon content (less than 60–70 Mg C/ha at 0–30 cm depth), they have the potential to increase soil carbon stocks. Additionally, planting biomass crops in degraded land can sequester carbon ranging from 0.6 to 3.0 Mg C ha-1 yr-1. The considerable benefits of biomass crops in sequestering carbon not only underscore their significance in mitigating climate change and reducing greenhouse gas emissions but also position them as vital crops for long-term carbon storage. The carbon stored has potential to then be sold in carbon credit markets.
Biodiversity enrichment
Biomass crops are beneficial for biodiversity compared with conventional cultivated areas of arable food crops. Several factors contribute to their positive impact, including longer rotation periods, low fertiliser and pesticide requirements, enhanced soil protection, greater spatial diversity, reduced disturbances during the growth phase, and the option to conduct harvesting during the winter or after the bird breeding season, minimising disruptions. Integrating biomass crops into agroforestry systems creates a diverse range of habitats and resources that attract various wildlife species. This combination of factors establishes an environment conducive to the survival and proliferation of diverse plant and animal species.
Biomass crops, such as willow and poplar, establish dense and varied stands that offer various niches and microhabitats. For instance, SRC willow contributes significantly to plant diversity when integrated into existing landscapes and agricultural systems. SRC willow plantations exhibit higher plant species richnessand increased weed cover compared to neighbouring arable agricultural lands. For example, a studyfound 27% greater plant species richness (133 flora species) and greater weed cover on SRC willow plantation when compared to the neighbouring arable agricultural land. Furthermore, a study into silvopastoral agroforestry, with 35-year-old poplar stands within grazed permanent pastures, found this interaction to offer a viable way of increasing the diversity of invertebrates, arthropods, and birds in grassland systems. The increased biodiversity within agroforestry systems with biomass crops contributes significantly to natural pest control. These ecosystems attract beneficial insects, such as ladybugs and parasitoid wasps, helping regulate pest populations. Consequently, this can reduce the need for chemical pesticides and promote more ecologically sustainable pest management practices.
Biomass crops, especially when integrated into agroforestry systems, provide valuable opportunities for fostering and preserving biodiversity, with positive effects often observed at the field scale. However, it is important to note that the impact on biodiversity within biomass plantations can vary significantly based on factors like plantation management, age, size, and heterogeneity.
Water and air quality improvement
Agroforestry systems with biomass crops can help regulate water flow, reducing surface runoff and the associated risks of flooding and water pollution. Biomass agroforestry systems, such as riparian buffersare recommended as a solution to combat nonpoint source pollution from agricultural land.
In areas where agricultural land is more intensively managed, nitrate and phosphorous leaching and run-off are identified as the main source of pollution resulting in eutrophication of lakes and waterways. Biomass crops can act as biological filters that take up and utilise nutrients before they enter watercourses. The crops have deep root systems that serve as safety nets, absorbing nutrients that have leached below the rooting zones of most arable crops.
For instance, a study showed increased nutrient removal (between 30-99% of nitrate and 20-100% of phosphorous) when biomass trees were incorporated into agricultural fields. Biomass crops such as willow SRC have been shown to be particularly effective for shown to be particularly effective for permanent nutrient removal of 55 kg PO43−eq ha-1 yr-1 based on field trials conducted in Northern Ireland.
Also, biomass crops (depending on their height and location within the landscape) can act as windbreaks and shelterbelts as well as protecting buildings and roads from snow drifts, and noise pollution. A mixture of both SRC and SRF in agroforestry system can offer the benefit of improving air and water quality.
Reduce soil erosion and improve soil health
The integration of biomass crops into agroforestry systems provides significant benefits for soil health and erosion control. Biomass crops have extensive root systems that help bind soil particles and prevent them from being washed away during heavy rainfall or strong winds. Biomass crop root systems reduce erosionby enhancing water infiltration, minimizing impacts by water droplets, intercepting rain and snow and stabilizing soil through their roots and leaf litter. Biomass crops have the potential to improve the soil’s physical, chemical, and biological properties by adding substantial amounts of above and below-ground organic matter, as well as the release and recycling of nutrients when integrated into agroforestry systems.
For instance, black locust roots form a symbiotic association with nitrogen-fixing bacteria, which form nodules on the roots and convert atmospheric nitrogen into ammonium in the soil, thereby enhancing soil fertility. When these crops are planted on marginal lands, they have the potential to rehabilitate and improve soil conditions. Black locust has been used successfully in mine reclamation in Germany and in reclaiming degraded lands in Romania. Studies have shown willow and poplar tree potential for use in the phytoremediation of soil contaminated with heavy metals.
Current UK policy
Biomass crops and SRF (<8-year rotation) are ineligible under current rules for woodland grants and incentives. There is therefore a need to develop policies that facilitate the integration of these crops into farming systems, acknowledging the environmental benefits provided by these crops, including improving soil health, carbon sequestration, enhancing biodiversity, and improving water and air quality. To encourage planting of these biomass crops on farms, grant options may be included in support schemes that farmers are already familiar with, such as the Environmental Land Management (ELM) Scheme in England, AgriEnvironment Climate Scheme (AECS) in Scotland, Sustainable Farming Scheme (SFS) in Wales, Small Woodland Grant scheme or the Forest Expansion Scheme in Northern Ireland, and the woodland grants and incentives available for establishing and maintaining woodland for nature recovery.
Further information:
Related Biomass Connect Articles:
- How does Short Rotation Coppice (SRC) willow affect biodiversity?
- What effect does planting biomass crops have on soil carbon?
- Biomass Buffer Strips – using biomass crops in multipurpose land management
Other resources:
- The Agroforestry Handbook
- Forestry Commission – Grant funding for woodland
- Funding for landowners – Nature based solutions to reduce flood risk
- Natural Flood Management Handbook – A practical guide for farmers.
Latest Technical Articles
Production of Switchgrass (Panicum virgatum) as a biomass feedstock
Key messages:
Switchgrass is a tall-growing, warm-season, perennial C4 grass native to North America. It grows in small dense clumps and is capable of producing high biomass yields with low inputs, grows well on marginal land, and tolerates a wide range of conditions.
Switchgrass is established from seed and has relatively low establishment costs compared to perennial biomass crops.
It takes approximately 2-4 years to mature and can remain productive for 15-20 years depending on crop management, soil type, climate and variety. Average yields of 7.57 to 12.72 dry tonnes per ha is achievable in the UK depending on management and harvest time.
Successful establishment requires attention to seed dormancy and weed control as well as proper depth and time of planting.
Switchgrass has several uses and benefits including control of soil erosion, forage for livestock, wildlife habitat and feedstock for biofuels.
Introduction
Switchgrass (Panicum virgatum) is a tall-growing perennial, warm-season (C4) grass native to North America. It grows in small dense clumps and is capable of producing high biomass yields with low inputs, and grows well on marginal or contaminated land and tolerates a wide range of conditions. Switchgrass consists of two ecotypes, the upland and lowland ecotypes. The upland ecotype has short fine stems and is more tolerant to drought and cold environments. The lowland ecotypes have thicker and taller stems and grow in wet areas with mild winter temperatures. They have late flowering and are adapted to warmer climates, but have lower drought and cold resistance than upland ecotypes. The lowland ecotypes are higher yielding compared to the upland varieties. The upland ecotypes however mature early compared to the lowland varieties.
Switchgrass grows to a height of 50 – 270cm. It takes approximately 2 – 4 years to mature and can remain productive for 15 – 20 years depending on crop management, soil type, climate and variety.
Uses and Benefits
- Switchgrass is a valuable soil protection cover. It is useful in soil erosion control and used in streamside buffers. It has a dense canopy and extensive network of roots which reduces raindrop impact, water runoff, and soil erosion.
- It is a good forage cover and habitat for a variety of wildlife. The canopy structure of switchgrass provides habitat for some birds, reptiles, amphibians, and mammals.
- The plant is an excellent forage for cattle and can be used as livestock bedding.
- It is used as an ornamental plant in gardens and landscaping.
- Switchgrass is a suitable feedstock for biodegradable plastics and for production of ethanol and butanol.
- Switchgrass has carbon sequestration potential and could reduce atmospheric Greenhouse Gas Emissions.
Establishment
Land management before planting in existing landscapes is important to scout for and control perennial weeds within the field to minimize stand failures in the establishment phase. Weeds, if not effectively controlled during the establishment phase, can result in crop failure. Switchgrass is planted by seed at a recommended depth of 0.5 – 2cm and at seeding rates of 200-400 pure live seeds (PLS) m-2. A seeding density of 5 – 15kg/ha is recommended depending on the variety and soil conditions. Switchgrass requires a firm smooth seedbed and can be planted by both no-till and conventional tillage. Conventional seed drilling provides more uniform stand development and seed depth.
Switchgrass displays a high level of dormancy which presents a challenge for germination. Breaking seed dormancy is important to reduce the risk of establishment failure and the costs associated with reseeding. Studies have reported various methods to break the seed dormancy of switchgrass including chemical, mechanical, thermal, and hormonal seed treatment. The seed dormancy of switchgrass is affected by the length of seed storage, storage conditions, quantity of seeds and soil conditions. It is recommended to conduct germination tests and treat seed dormancy by stratification (i.e., soaking seeds in water for about 1 month at 5-10oC then re-drying or by after-ripening (i.e., holding seeds at moderate or preferably at elevated temperatures until the dormancy is broken).
The ideal time to plant switchgrass is early spring after the last frosts (May to mid-June) when soils are moist, and the root system has plenty of time to establish. Switchgrass is a warm-season grass and requires a soil temperature above 10oC for satisfactory germination.
Rolling is recommended both before and after sowing to conserve the moisture in the seedbed followed by the application of broadleaf herbicide to control weeds. Weed competition is a major reason for switchgrass stand failure, especially during establishment. It is important to control weeds to avoid competition. Soil testing is recommended before planting to determine recommended fertiliser application rates to apply. Depending on the soil, nitrogen fertiliser application may not be necessary during the planting year as this will encourage weed growth and increase the risk of switchgrass being outcompeted by weeds as well as increase the establishment cost.
Site Suitability
Switchgrass establishes best on well drained, fertile loam or sandy loam soils, but it can establish and persist under highly variable soil conditions. Switchgrass can be grown on marginal land not suitable for food and feed production and it will tolerate short periods of waterlogging and, depending on the variety, has good tolerance of heat and drought stress. A study investigated different varieties of switchgrass for adaptation in Europe and found that the main factors determining the adaptation of varieties is latitude of the origin of the variety.
Biomass production and harvesting
Average yields of 7.57 to 12.72 dry tonnes (t) per ha are achievable in the UK depending on management and harvest time. A study in the UK of a 2-4 year trial across four sites found lowland varieties (NL93/2) to have a yield potential of 9.63 t ha-1 yr-1, and upland varieties (Shelter and Cave-in-rock) to have yields of 7 t ha-1 yr-1. Switchgrass compared to other C4 grasses like Miscanthus (Miscanthus x giganteus), has a relatively lower yield. Miscanthus average yields of 22 t ha−1 yr−1 have been observed in Europe and the US compared to 10 t ha−1 yr−1 of Switchgrass. In areas with sufficient rainfall, sustainable yields of ∼15 Mg ha−1 yr−1 may be achievable by applying ∼50 kg N ha−1 yr−1. In South England, the dry matter yield of switchgrass (Cave in Rock variety) grown on low productive arable crop land increased by 43% by the application of 100 kg ha-1 Nitrogen fertilizer.
Mature switchgrass can be harvested once or twice per year with conventional haying equipment and usually commences from the second year after sowing. Harvesting can be conducted after frost when plants have senesced and translocated nitrogen and potassium back into the root system. Harvesting once per year will remove fewer nutrients from the soil, maximize biomass recovery and maintain stands. Harvesting twice per year will require more nitrogen application to compensate for the nitrogen removed in the first harvest. Harvesting more than twice a year adversely affects long-term productivity and persistence of the crop. Switchgrass traits can be significantly affected by the harvest timing.
Switchgrass is suitable for thermochemical processes such as combustion, gasification, and pyrolysis. A study found switchgrass composition of lignin (12.3), cellulose (46.1), hemicellulose (32.2), ash (4.7) to be suitable for thermochemical processes, hence it is an attractive feedstock for bio-based products.
Feedstock | Cellulose | Hemicellulose | Lignin | Ash |
Miscanthus | 41.9 | 26.6 | 13.3 | 3.2 |
Switchgrass | 46.1 | 32.2 | 12.3 | 4.7 |
Wheat straw | 34.9 | 22.5 | 21.3 | 9.4 |
Rice straw | 41.6 | 31.5 | 12.5 | 14.4 |
Corn stover | 39.4 | 33.1 | 14.9 |
|
Sorghum | 15.0 | 12.3 | 5.8 | 0.4 |
Sugar cane | 48.6 | 31.1 | 19.1 | 1.2 |
Hardwood (beech) | 43.3 | 31.8 | 24.4 | 0.5 |
Softwood | 40.4 | 31.1 | 28.0 | 0.5 |
Source: Adapted from Pauly and Keegstra (2008)
Pests and Diseases
Switchgrass disease levels are relatively low and not considered a cause for concern. Fungal diseases such as rust, leaf spot and smut have been reported to affect switchgrass. Varieties developed in dry areas will be more susceptible to fungal disease when grown in humid conditions. The lowland ecotypes are known to be more resistant to rust. Aphid, leaf miners, double-lobed moths and fruit flies occasionally attack switchgrass causing a reduction in yield. Damage from rabbits and slugs can occur during the first year of establishment.
Economics
Switchgrass has a relatively low establishment cost compared to other perennial grasses like miscanthus, and other biomass crops like willow and poplar. Switchgrass is cultivated by seed and has low input cost (around £357/ha), thus the establishment is of low cost. Where production costs have been considered, research indicates that production cost of switchgrass in some European countries including UK indicated values from 130 to 250 £/ha/year. This makes it a practical option among biomass crops. There is little data available on the commercial production of switchgrass in the UK. Most existing plantings are for research purposes.
Latest Technical Articles
Reed Canary Grass as a Biomass Crop
Key messages:
- Reed canary grass is a perennial, lignocellulosic crop that is native to the UK and has multiple uses.
- It is established from seed, making set-up costs lower than for many other perennial biomass crops.
- Reed canary grass is a shorter-term crop (5-10 years) than Miscanthus and willow, with average yields of 5-7 tonnes of dry matter per ha, depending on management.
- It is tolerant of a range of land types and conditions including flooding, drought, and freezing.
- Reed canary grass can be grazed in early summer (depending on the variety) and harvested for diverse other uses from late summer until early spring. This extends the annual window of biomass availability and supports multiple end uses (e.g. anaerobic digestion, combustion, animal bedding, seed supply, game cover).
Introduction
Reed canary grass (Phalaris arundinacea L.) is a multipurpose, lignocellulosic perennial crop native to Europe, Asia, and North America. It can be grown on poorly productive land and tolerates a wide range of conditions. Reed canary grass is found in diverse habitats including wetlands and riparian zones but also drier areas like roadsides, forest margins, pastures, and disturbed areas. It is a fast-growing crop reaching heights of 1.5-2 m in a growing season, and, depending on management, can remain productive for 5-10 years.
Uses and Benefits
Reed canary grass can be utilised in diverse energy conversion processes like combustion, gasification, pyrolysis, and anaerobic digestion.
- Reed canary grasshas a high gross calorific value (dry ~18.2 MJ kg-1), exceeding that of wheat and Miscanthus. Ash content (~3-8 %) depends particularly on harvest, management, and site.
- Reed canary grass has carbon sequestration potential and can be used for a prolonged ‘fallow’ period in a rotation where soil health can recover from more intensive arable practices and organic matter can be accrued.
- Reed canary grass creates habitat for diverse wildlife including birds and small mammals.
- Reed canary grass can mitigate greenhouse gas emissions and reduce nitrate leaching, acting as a buffer crop.
- Reed canary grass can be integrated into multifunctional landscapes as a biomass crop providing benefits of supporting ecosystem services and mitigating environmental impacts.
- Reed canary grass can be used for livestock feeding provided it is low in alkaloids (eg. Marathon or Palaton variety). It has good absorption properties and is a viable alternative to straw as bedding material for livestock.
- Reed canary grass has potential to remove contaminants from soil and water.
Establishment
Reed canary grass is planted by seed. It requires a firm, smooth seedbed and timely weed control during establishment, with fertiliser where nitrogen is limiting. Seed can be broadcasted or planted using a conventional seed drill. Conventional seed drilling provides more uniform stand development and seed depth. The ideal time to plant Reed canary grass is between March and April when soils are moist, and the root system has plenty of time to establish. The recommended seeding rate for forage production is 5-10 kg ha-1 with row spacing of 12.5 cm, at a depth of about 2 cm. Rolling is recommended both before and after sowing to conserve the moisture in the seedbed followed by application of broadleaf herbicide to control weeds. Growth can be slow during the first year of cultivation while the root and rhizome system establishes. Harvesting can usually commence from year two. Nutrients translocate to the rhizomes over the autumn and winter period, providing energy for new shoots in spring, and improving the sustainability of the crop and quality of the biomass for combustion purposes.
Site Suitability
Reed canary grass is highly tolerant to waterlogging (established stands can tolerate extended periods of inundation) and a wide pH range from pH 4.9 to pH 8.2. It can thrive on marginal land unsuitable for food or feed production. Once established, Reed canary grass is one of the most productive temperate grasses during drought due to its deep rhizome and root system. As a temperate grass used extensively in Scandinavia, it is also highly tolerant of freezing conditions.
Biomass production and harvesting
Average yields of 5-7 tonnes per hectare are achievable in the UK, depending on management, although ‘pushing’ the crop may decrease crop overall persistence. Yields will be improved by fertilisation where nutrients are limiting although over fertilising may promote lodging. Harvesting the crop green will reduce sustainability, as nutrients not yet translocated to the below-ground biomass will be removed.
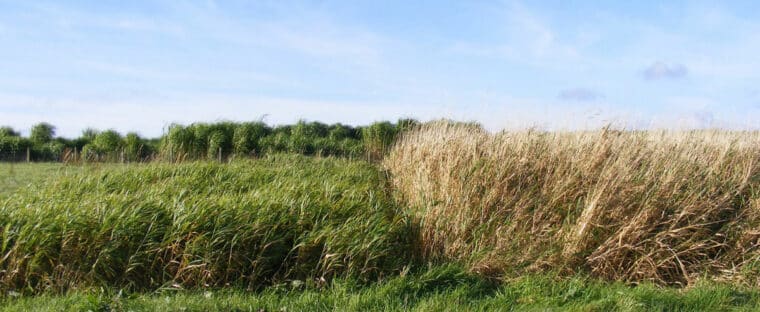
Reed canary grass under two harvesting regimes (autumn, LHS; spring RHS) on shallow, stony soil in West Wales.
Harvesting can be conducted with conventional grass harvesting machinery and usually commences from the second year after sowing.
The timing of harvest depends on the intended market/end-use. Forage quality is best in spring and early summer, while harvest for anaerobic digestion can extend from summer until autumn senescence begins. For combustion and animal bedding, harvesting is best delayed until late winter or early spring, where combustion quality will be improved through nutrient translocation below ground, leading to lower contents of sodium, potassium, chlorine and moisture (ca. 10-15%). Spring-harvested biomass requires little or no drying. The crop is typically mown first before being baled and transported.
Alternatively, the grass can be cut and chipped using a forage harvester. Eliminating the crop after productive years will be easiest using herbicides e.g., glyphosate, although repeated cutting and conventional tillage may suffice.Although harvesting reed canary grass green will remove nutrients, increasing the need for fertiliser, research has shown costs can be reduced using sewage sludge or nitrogen-fixing legumes.
Pests and Diseases
Reed canary grass disease levels are relatively low and are not considered a cause for concern (they include brown rust, mildew, buff spot, powdery mildew, and Rhynchosporium (leaf scald)). However, reed canary grass can be attacked by insect larvae, which feed inside stem bases and can occasionally significantly reduce yield. Damage from rabbits and slugs can occur during the first year of establishment.
Economics
Returns during the first year are low, as establishment costs will not be balanced by revenue from biomass production. A study conducted in the UK found an average gross margin of £153.89 ha-1 for reed canary grass grown in multiple sites. The gross margins ranged from a minimum of £110.60 ha-1 to a maximum of £219.40 ha-1 across the sites studied. The differences in the gross margin were attributed to the variations in yield among different sites and prices of the biomass.
Latest Technical Articles
Does production of biofuel mean less food production?
Take home messages:
- The food versus fuel debate calls into question the ethics of diverting land from food to biofuel production.
- Bioenergy production from first generation (edible) biofuel feedstock may compete with food production and as consequence affect food security.
- A shift towards increasing production of second generation (non-edible) biofuel feedstocks and third generation biofuels along with advanced technologies of genetically engineered algae biomass would avoid the competition for food.
- Food and bioenergy need not compete for land. Biofuel crops should be integrated into existing landscape and agricultural lands in a multifunctional approach to improve use as food, fuel and for other ecosystem services.
Introduction
Biofuels have the potential to reduce reliance on fossil fuels whiles reducing environmental impacts and greenhouse gas emissions. Biofuel makes a small contribution to our energy demand whiles fossil fuels contributing approximately 87 percent of energy having a negative impact of increase in global warming. To limit global warming, biofuel production should be increased from 9.7 × 106 GJ d−1 to 4.6 × 107 GJ d−1 between 2016 and 2040. This would require large scale deployment of biofuel feedstock production and advanced technologies in biofuel production. However, there are trade-offs related to the expansion of bioenergy feedstock production by diverting land use from food to biofuel production, which poses a threat to food security especially in developing countries, and directly or indirectly leading to deforestation and other changes of land use that have a negative effect on greenhouse gas emissions. This calls into question the ethics of diverting land from food to energy and whether it implies less food production. In this article, we provide a review of the food versus fuel debate, whether a shift to other alternative biofuel feedstocks would avoid this competition for food.
Food versus fuel debate
The food versus fuel debate calls into question the ethics of diverting land from food to energy production. The opinion piece written by the Guardian columnist, George Monbiot in 2004, brought attention to the issue of devoting land to fuel production in his article entitled ‘Feeding cars, not people’. He argued that the expansion of biofuel production is at the expense of food which is much needed to feed a growing population especially the food insecure in developing countries. A wide range of perspectives on the debate of food versus fuel further emerged following increases in world crop prices for cereals and vegetable oils which are raw materials for producing biofuels. Biofuels are produced from the fermentation of biological feedstocks containing fermentable sugars, lipids, or carbohydrates. Biofuels are categorized into first, second, third and fourth generation biomass feedstocks (see figure 1).
What are the implications of use of first generation feedstocks for biofuels?
First generation biofuel feedstocks have been at the centre of the food versus fuel debate. First generation biofuels are produced from easily accessible and edible fractions of food crops. They produce large amounts of lipids or carbohydrates that are easily converted into biofuels. Bioethanol is produced from sugar crops (e.g., sugarcane, sugar beet and sweet sorghum) and starch crops (e.g., wheat, maize, and cassava), while oil seed crops such as rapeseed, sunflower, oil palm, and soybean may be used for production of biodiesel. These edible feedstocks have been mainly used for producing biofuels globally and have been highly commercialised, contributing over 50 billion litres of the total fuels produced per year. However, there are trade-offs with producing such edible feedstocks for biofuel production.
First, increasing production of first generation biofuel feedstock impacts food security, due to competition for direct land use. Thus, any dedicated use of productive land for production of biofuel feedstock inherently comes at a cost of not using the land for food or feed production. This claim is however based on the assumption that land is a limiting factor for global food production and to meet the rising demand for food and fuel will require newly cleared land leading to deforestation and increases in greenhouse gas (GHG) emissions. On the other hand, others have argued that global land is not a limiting factor when land cover, land use, and productive potential are considered.
Another facet to the debate is that the demand for biofuels has an impact on food prices, which disproportionately affect poor people in the global south who spend roughly half of their household incomes on food. The increased demand for production of biofuels from food grains and oilseeds in the U.S. and the EU contributed to this increase in food price, much of which was due to policies that incentivised production of biofuels. On the other hand, some studies have shown that biofuel prices do not seem to affect food commodity prices and have not been the most important contributing factor for food price inflation and that, different biofuels have different impacts on prices of food commodities. Such studies have found no strong evidence that biofuels such as ethanol would drive food commodity price.
Furthermore, there is evidence that these biomass feedstocks may not have a direct impact on food production since they are grown for different purposes. For instance, from the data on global use of major food commodities (see figure 2), the volume of use of maize, a major biofuel feedstock is mostly used for animal feed. The OECD-FAO projections on global use of maize is largely more towards animal feed production. Thus, the type of biomass production may not have a direct impact on food production since they are grown and used for different purposes. Hence, there is the need to examine the interdependencies between the multiple end-uses of biofuel feedstocks.
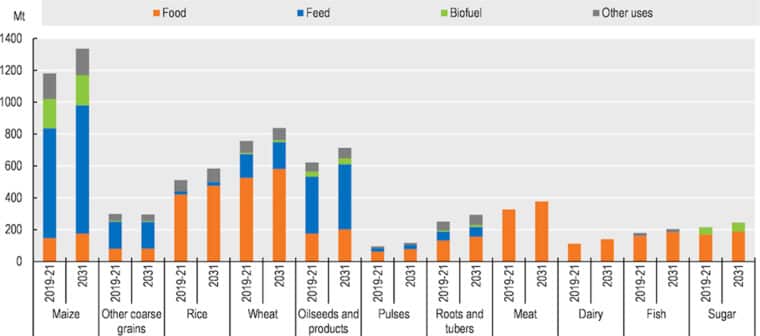
Figure 2: Global use of major commodities
Lastly, the studies have shown that first generation biofuels are poor crops for biodiversity and compete for water resources. For example, production of maize presents the risk of soil erosion, soil damage and runoff water which affects soil health. Limits should be set on bioenergy support schemes to regulate or control the amount of food and feed crops that can be used for production of biofuels.
Would second generation biomass crops avoid the competition for food?
In view of the negative trade-offs of first generation biofuel crops, second generation biofuel feedstocks which are derived from non-edible biomass have been proposed as more suitable for production of biofuels, as these feedstocks do not compete with food production. Second generation biofuels are based on lignocellulosic feedstocks including perennial energy crops (e.g., Miscanthus, short rotation coppice willow, poplar), Short rotation forestry (e.g., eucalyptus, black locust, paulownia), agricultural residues (e.g., forest thinning, sawdust, sugarcane bagasse, rice husk, rice bran, corn stover, wheat straw, and wheat bran), and forestry residues (e.g., small branches and bark from forest thinning operations, conservation management operations, wood pellets, or wastes from wood processing industries such as sawdust).
Compared to first generation biofuel feedstock, these non-edible feedstocks do not compete for food, can grow on marginal land, do not require much input such as fertilizers, and provide additional ecosystem benefits such as increasing biodiversity and soil carbon and reducing greenhouse gas emissions. They produce high amounts of biomass and can be harvested regularly over a long period and be processed for use as biofuels. These biomass crops have a potential to play a key role in decarbonisation of economies, both as a substitute to fossil fuel energy reducing greenhouse gas emissions, as well as to generate negative emissions using carbon capture and storage (BECCS).
However, the competition for land and food may still persist if productive land for food production is used for growing second generation biomass feedstocks. Planting second generation biomass feedstocks on marginal land avoids the competition for land use and food. Also, bioenergy crops should be integrated into existing landscape and agricultural land in a multifunctional approach to offer multiple benefits including use as fuel and other ecosystem services.
Shifting to third generation bioenergy feedstock and more advanced technologies?
Third and fourth generation biofuels are more promising as they do not create such land use and food competition. Third generation biofuels are non-food marine biomass derived from algae including, seaweeds (green, red, and brown macroalgal species) and microalgae. These algae biomass have higher growth rates and do not require an arable or big land area for production and hence do not compete with land for food and also act as carbon sink.
With advanced technologies of genetic modification of microalgae, fourth generation biofuels are seen as more promising sustainable biofuel feedstock due to their high biofuel productivity and ability to capture large amounts of carbon dioxide. Research is still advancing in the use of genetically modified algae. As these technologies become more advanced, efficient, and economical, they could replace uses of first generation biofuels and fossil fuels for sustainable energy production.
Sustainable biofuel production in the UK
In the UK, bioenergy plays an important role in decarbonising the energy system by reducing greenhouse gas (GHG) emissions, expanding renewable energy and moving towards a low-carbon economy. Domestic biofuel crop production however constitutes a small component of existing agricultural land. Less than 1.4 percent of 18.6 million ha of agricultural land in the UK is currently used for bioenergy crops. In 2020, 121,000 ha of agricultural land were used to grow bioenergy crops. Of this, 92 percent constituted edible food crops for biofuels (diesel and bioethanol) and biogas, and about 8 percent constituted non-food biofuels used for electricity and heat generation. Future projections to reach net zero ambitions estimates planting about 30,000 ha yearly through to 2035 and estimated cultivation of 70,000 ha of bioenergy crops by 2050. This will certainly require large scale expansion of land for biofuel feedstock production.
A shift towards increasing production of second generation (non-edible) biofuel feedstocks and third generation biofuels along with advanced technologies of genetically engineered algae biomass would avoid the competition for food. The second generation biofuel crops may not necessarily compete directly with food production if grown on marginal lands including under-used, inaccessible, inconvenient, land prone to flooding, or degraded land. It is estimated that 1.4 million hectares of marginal land in the UK could potentially be planted with perennial energy crops by 2050 avoiding competition for arable land for food production. Planting should also be prioritised on low quality agricultural lands (Grade 5‐4‐3b), public access land, contaminated land and land identified by the Forestry Commission as low risk for woodland creation. However, planting should be avoided on UK Biodiversity Action Plan (BAP) priority habitats, land with cultural value, peatlands and other high carbon soils >120 t C ha‐1.
Food and bioenergy need not compete for land and, instead, should be integrated to improve resource management. Bioenergy crops should be incorporated into existing landscape and agricultural land in a multifunctional approach to deliver multiple outputs from the land including food, biodiversity, climate change, waste absorption, and other societal purposes.
Conclusion
The dilemma of diverting land from food to energy production has made bioenergy crops controversial with wide range of perspectives. Biofuels produced from using edible biomass stocks have been at the centre of the food versus fuel debate, with serious criticism that it may lead to competition with food, increase food prices, deforestation and other changes of land use that have a negative effect on greenhouse gas emissions. A shift towards increasing production of second generation (non-edible) biofuel feedstocks and third generation biofuels along with advanced technologies of genetically engineered algae biomass would avoid the competition for food. Planting second generation biomass feedstocks on marginal land avoids the competition for food production. Food and bioenergy need not compete for land, instead, biofuel crops should be integrated into existing landscape and agricultural lands in a multifunctional approach to offer multiple benefits including use as fuel and for other ecosystem services.
Latest Technical Articles
Hemp as a Biomass Crop
Take home messages:
- Industrial hemp has greater versatility and profitability than many other biomass crops like giant miscanthus, willow, poplar and switch grass.
- It yields high biomass (12- 15 t/ha of air-dried biomass) with minimum input of water, fertilizers and pesticides.
- The best applications for industrial hemp include co-production of hemp products like insulation material for buildings, hemp paper, textiles etc with biofuels.
- It is an excellent rotation crop that fits well with food and feed crops and improves the yield of the subsequent crops due to the beneficial effects of hemp on the soil like improving soil structure and reducing parasitic nematodes and fungi.
- The UK lags behind its European counterparts in hemp production due to the regulatory forces and unless changes to these regulations are made, farmers may be less attracted to the produce hemp.
Introduction
The conversion of biomass to biofuels has gained heightened interest due to the increasing demand for sustainable and eco-friendly sources of energy. Traditionally, biofuels have been produced from starchy or sugar crops such as corn, wheat, sugar beets and sugar cane. However, this gives rise to competition between food versus energy. Therefore, there is a need for dedicated non-food energy crops, especially those where the whole plant biomass can be used for energy production resulting in potentially higher land use efficiency. Moreover, if such crops could be fitted into a crop rotation system with food and feed crops land use efficiency can be further increased. Energy crops are projected to play a significant role in the future of bioenergy due to the paucity of biomass from forestry output and the scarcity of acceptable “waste” streams.
Industrial hemp (Cannabis sativa L.) is one of the oldest crops in the world traditionally grown for its fibre. Hemp fibre was the main fibre for maritime ropes and sails and was used extensively as a raw material for cordage and textiles. However, its production declined in the middle of the 19th century due to the disappearance of the sailing navy, competition from natural fibres like cotton and jute and later due to intensive development of synthetic fibres.
In the 1930s cultivation was forbidden in most Western countries and in North America due to its genetic closeness to marijuana which created a lot of confusion and social, political and moral controversies. The 1990s marked renewal of hemp cultivation for agricultural, industrial and scientific reasons throughout the world mainly due to increasing consideration of natural resources, energy conservation and biomass conversion to bioproducts and biofuels.
Since 1992, France, the Netherlands, England, Spain and Germany have passed legislation allowing for the commercial cultivation of industrial hemp. Two years later, Canada followed in the footsteps of the EU passing regulations that allowed farming of hemp. The area dedicated to hemp cultivation in the EU (fig 2) has increased significantly from 19,970 hectares (ha) in 2015 to 34,960 ha in 2019 (a 75% increase). In the same period, the production of hemp escalated 62.4% from 94,120 tonnes to 152,820 tonnes. France is the leading producer in Europe, accounting for more than 70% of EU production, followed by the Netherlands (10%) and Austria (4%).
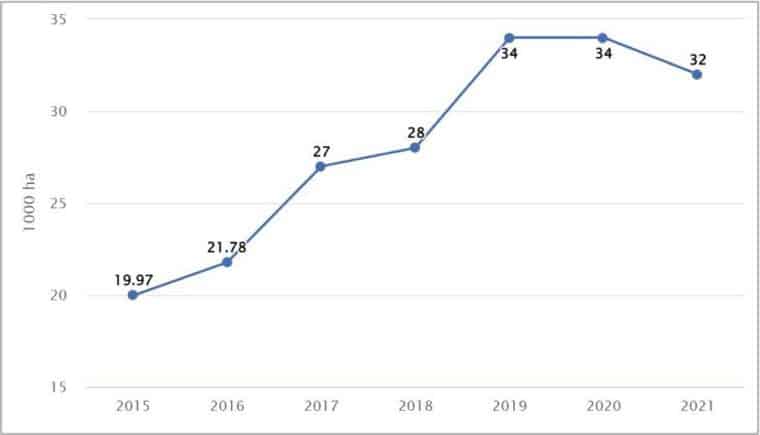
Fig 2. EU land used for hemp Fig 2. EU land used for hemp cultivation from 2015- 2021cultivation from 2015- 2021
Recently there has been renewed interest in hemp as an insulating material as well as a feedstock for specialist paper. It is also an excellent rotational crop. It uses a fraction of the water needed to grow cotton, absorbs more carbon dioxide per hectare than other crops and most trees and its every part is beneficial. Its extensive root system improves soil structure and subsequent crops have less weed pressure. Studies in winter wheat crops grown after hemp have reported yield increases of 10-20%. This may be attributed to decreased parasitic nematodes and pathogenic fungi and reduction in soil water loss and soil erosion due to hemp growth.
Hemp is also highly efficient in ecological reconstruction and land reclamation owing to its phytoremediation capacity i.e its ability to remove heavy metals from the ground. Overtime it can remove heavy metals and other contaminating substances from the deeper layers of the soil as its root system develops. Finally, biochar produced from hemp for soil applications could potentially improve soil carbon sequestration and reduce greenhouse gas emissions.
Important factors in growing hemp for Energy
Soil and Climate
Hemp cultivars adapt well to a temperate climate therefore they can be farmed in most countries in Europe including UK. During the growing season, they require 200–300 mm of rainfall. The amount of rain that falls throughout the summer is less crucial for development than enough soil moisture. A strong tap root system effectively lessens the impact of a brief drought. As a result, seed germination can take up to 8–12 days at a soil temperature of 8–10oC. Young plants may withstand brief frosts of up to -6oC degrees. The duration of the day has a significant impact on how hemp develops. Hence, the recommended time for planting hemp for biomass is at the end of April.
A well-established soil that is nutrient- and organic-rich is necessary for hemp. The soil should have a pH that is neutral or slightly alkaline. A robust root system ensures that plants can absorb water and nutrients from the soil’s deeper layers. In crop rotations with a large proportion of cereals, hemp is a desirable preceding crop because it enhances the aggregative structure of the soil.
Tillage and Cultivar Selection
Hemp requires winter ploughing and spring tillage to prevent soil dryness. The amount of nutrients in the soil and the previous crop should be taken into consideration when deciding how much mineral fertiliser to apply before planting. The amounts of specific fertilisers are as follows: approximately 80 kg/ha of N, 50 kg/ha of P2O5, and 90 kg/ha of K2O. In general, hemp doesn’t need chemical pesticides or weed killers to control weeds, diseases, or pests because it has one of the intensive early growth rates of any agricultural crop. Hemp should be planted at a density of 40–50 kg/ha with a row spacing of 20–30 cm.
There are 75 varieties listed in the EU common catalogue of varieties of agricultural plant species. The main factors that influence the choice of the hemp variety include the suitability for growth in the region and the end product for which it is grown. Most cultivars of hemp are capable of yielding 12–15 t of air-dried mass per hectare genetic and breeding work is carried out to obtain new lines yielding over 20 t/ha of biomass with a high fibre and cellulose content and trace amounts of THC.
What makes hemp a potential bioenergy crop?
The demand for biomass for conversion to energy is rapidly increasing and is expected to accelerate in the coming years. Industrial hemp is valuable due to its high biomass and energy yield per hectare. Several studies have claimed that hemp could be used in energy production as a fuel source with no sulphur emissions either by direct combustion or converted into liquid fuels such as bioethanol. Hemp oil, which was traditionally used as lamp oil, can be used to produce renewable biofuels which emit less carbon monoxide on combustion to replace gasoline for diesel engines thus helping in relieving global warming.
The whole plant can be used to make a variety of products in a biorefinery, including feedstock and biochemicals like succinic acid, heat from briquettes or pellets, electricity from baled biomass and vehicle fuels like biogas from anaerobic digestion or bioethanol from fermentation. In terms of biofuels, hemp can provide, biodiesel made from the pressed seed and bioethanol and methanol from the fermented stalk, all of which are clean renewable alternatives to petroleum-based fuels. The possible pathways for bioenergy production from hemp biomass are illustrated in figure 5.
Hemp is a rapidly growing plant that can withstand high planting density and its total biomass per hectare is similar to other energy crops like the giant miscanthus, poplar, switchgrass and willow. However, the significant advantage of hemp over other energy crops is that the concentration of digestible cellulose and hemicellulose is higher in hemp bast fibres. They contain 73-77% cellulose, 7-9% hemicelluloses, 2-6% lignin compared to 48%, 21-25% and 17-19% respectively in the hurd. On the other hand the cellulose and hemicellulose of hemp hurd are comparable to that in the stems of giant miscanthus, poplar, switch grass and willow (table 1). Notably, 20–30% of the stem biomass in hemp consists of high cellulose fibre, resulting in a higher ratio of digestible sugars to lignin in hemp than in other similar-yielding biofuel crops. These characteristics make hemp an above-average energy crop for some biochemical-based biofuel production and greenhouse gas mitigation applications.
Table 1. Fibre yield and composition of hemp compared to other proposed biomass crops.
Crop | Biomass (Mg DM/ha/yr) | Cellulose (%) | Hemicellulose (%) | Lignin (%) |
Hemp fibre | 7 – 34.0 | 73-77 | 7-9 | 2-6 |
Hemp hurd | 34-48 | 21-25 | 17-19 | |
Corn stover | 4.6 – 5.5 | 38 | 26 | 17 |
Switchgrass | 5.4 – 34.6 | 28-37 | 23-27 | 15-18 |
Giant miscanthus | 10.0 – 44.0 | 50-52 | 25-26 | 12-13 |
Poplar | 7.7- 17.3 | 42-49 | 16-24 | 21-30 |
Willow | 6.2-21.5 | 46-56 | 12-14 | 13-14 |
Fuel Quality of Hemp
The most important characteristics when evaluating any fuel are the calorific value, ash content, melting behaviour, physical handling characteristics, and any negative effects on heat exchanger corrosion or stack emissions. The following section assess the fuel quality of hemp. Comparison to other fuel crops is made where data is available.
Calorific value
This is the most important property of any fuel. Biomass from both the whole hemp plant as well the by-products (shives and straw) can be a good raw material for energy production. A study at the Teagasc biofuel laboratory reported that, provided hemp can be dried in the field to a moisture of 20%, its net calorific value is higher than many other forms of biomass and much higher compared to that of peat (table 2). Thus, hemp can be an excellent alternative to plants operating on peat fired energy. However, differences in gross caloric value are small.
Table 2. Gross and net caloric values of some biomass fuels.
Material | Calorific value (MJ/kg) | Assumed moisture content (%) | |
Gross | Net* | ||
Hemp | 18.5 | 13.4 | 20 |
Wood residues | 19.7 | 10.0 | 40 |
Straw | 18.0 | 13.0 | 20 |
Peat | 21.5 | 8.9 | 50 |
*heat available with no further drying and no recovery of latent heat
Another study reported the gross calorific values of hemp whole plant and by products compared to other biomass and found them to be comparable or better (fig 6). This study also reported hemp to be resilient to conditions of draught and the energy efficiency per hectare of hemp exposed to water shortage was 10% higher than that of maize under the same conditions confirming the suitability of industrial hemp for energy production.
Ash and Melting Point
The ash content of hemp samples reported in scientific literature varies between 3-4% which is much higher than wood but lower than peat and similar to many non-wood biomass materials. Hemp’s high ash content unavoidably extends boiler maintenance times and causes ash disposal issues but how much longer depends on the application. For instance, it might render the fuel unusable as a pellet feedstock for home heating stoves or boilers in urban areas, be anticipated to have little impact on the rural boiler market and be welcomed by generating stations already using peat with 6-7% ash.
The melting behaviour of ash is also crucial, particularly in applications requiring high combustion temperatures. Low melting temperatures can cause ash to clump together and obstruct grate openings and ash removal systems. The melting behaviour depends on the composition of ash but even with variations the calculated values for hemp ash melting point is higher than most other non-wood biomass. Therefore, under normal combustion conditions ash melting problems should not be an issue with hemp.
Corrosivity and Emissions
One of the major issues with non-wood material is the propensity of the fuel to corrode heat exchanger surfaces and is mainly a function of chlorine, potassium and sodium contents of the material. Hemp has low Cl and Na levels, while its K content is higher than that of wood but similar to that of many straws. Hemp typically has superior qualities to cereal straw, which is effectively burned in power plants in Denmark and the UK, and boilers and Combined Heat Power (CHP) plants in Denmark.
Stack emissions (gases released into the air from boiler stack, chimneys or diesel generator set stack) depend on the plant design, operational factors as well as fuel composition. A fuel with high volatile content and low in sulphur, chlorine, potassium and heavy metals generally tend to have low stack emissions. Hemp stems contain <80% volatile compounds higher than most other plant biomass and moderate levels of S, Cl and K. Although no excessive heavy metals have been measured in hemp, but as hemp as the ability to take up heavy metals from the soil, emissions may be a problem if hemp is grown on contaminated soils.
Profitability analysis
The main factors to consider when determining profitability include crop yields, production costs, energy costs, and the support available for renewable energy. The following section analyses the profitability of hemp grown for energy based on these factors.
Yield
Hemp’s ability to produce significant biomass yields with relatively little water input is one of its main advantages. Hemp requires less water than many other crops, including alfalfa and maize, and may produce 15 t of dry matter with only 250–400 mm of water when grown using modern production techniques. The ambient temperature is another factor that influences hemp development; it can be grown most successfully between the ranges of 13 and 22 °C. As hemp has natural defences against disease and insect attack, growing it requires just a little amount of biocides. These factors make hemp relatively inexpensive to grow.
A 3-year study in Ireland showed that under Irish climatic conditions early sowing has a big impact on yield. Furthermore, seed rate can be chosen to optimize yield and seed cost without any concern about fibre quality. This study also reported that a seeding rate of 30kg/ha gave the highest yields. The study concluded that a harvested yield of 11 t/ha of dry matter, corresponding to about 13.7 t/ha at 20% moisture is achievable and profitable.
Harvesting
The cutting and windrowing procedure is expensive and has a significant impact on field losses, in-field drying, and baling efficiency. There is a need to develop new harvesting machine designs that enable the co-production of improved hemp products, such as high-quality seed and straw which may in turn help to improve the viability of industrial hemp production.
Economics of growing hemp
Hemp grown for energy must be profitable for farmer to produce biomass for energy. In order to ensure encouraging prices for the finished product based on contracts, lower production costs are required. The low-cost production of fibre, seeds, or essential oil for technical uses can slightly increase the profitability of hemp produced for energy. The residual biomass (straw and shives) can subsequently be sold as briquettes for use in the generation of energy.
Hemp production varies greatly on an annual basis, and therefore the profitability of growing hemp inevitably changes as well. In Canada, one of the top hemp producers in the world, the revenue from hemp production is estimated to be $510/acre for fibre, $400/acre for grain, and $580/acre for both fibre and grain hemp production. This is comparable to the suggested returns from a US study.
Another US study which compared hemp with kenaf, switch grass and sorghum found that hemp generated the greatest revenue of these four crops, yielding $2632/ha ($2462/ha from grain and $170/ha from ethanol) compared to $908 for kenaf, 4803/ha for switchgrass and $1725 for sorghum when used for coproduction of ethanol and grain, though hemp had lower biomass yield. These values include the yield and value of hemp and sorghum grain as well as the growing cost for each type of biomass. One study looked at the profitability of hemp by pricing it as an energy commodity with the same price as oil. It found that industrial hemp had a net yearly profit of between €2000 to €3500/ha, which was higher than the earnings from both canola and sugar beetroot. Using organic fertilisers also enhanced profits, though less significantly than for canola and sugar beetroot.
In EU countries, farmers who grow hemp are eligible for area-based direct payments under the Common Agricultural Policy (CAP) provided the hemp variety being cultivated has THC (Tetrahydrocannabinol, the main psychoactive compound in marijuana) content of no more than 0.3% and is listed in the EU common catalogue of varieties of agricultural plant species. In the UK very little hemp farming has taken place in the recent years, in spite of a long hemp farming history, and the nation today falls behind its European rivals significantly. Only 20 farmers in the UK currently cultivate hemp on an area of 800 hectares. The main cause of this is the onerous regulations. Industrial hemp cultivation is legal in the UK with a licence since it is considered a “special purpose” under the Misuse of Drugs Act of 1971 (MDA). As a result, hemp farmers must adhere to strict guidelines for the licensing of controlled substances.
Conclusion
Hemp has great potential to become a promising commodity crop for producing both biofuels and value-added products. It is an excellent rotation crop that fits well with food and feed crops and improves the yield of the subsequent crops due to the beneficial effects of hemp on the soil. The best applications for industrial hemp include co-production of hemp products with biofuels. Its high yield, higher ratio of cellulose to hemicellulose and digestible sugars to lignin and economics of production give it a competitive advantage over other biomass crops like giant miscanthus, willow, poplar and switch grass.
To promote the development of hemp as a biomass crop in the UK, changes to the regulations which require licensing to produce hemp are required. Subsidies for investments in processing facilities and equipment would greatly increase the viability of hemp in existing crop cycles in the grasslands, where hemp biochar can be co-fired with coal to reduce emissions. Hemp producers can benefit from investment in processing equipment that can valorise many potential products from hemp.
Latest Technical Articles
Harvesting of Short Rotation Coppice Willow
Summary
Willow is harvested every three to four years depending on the plant growth and soil conditions. Ideally, harvesting should occur during the dormant season in winter (mid-October to early March) after leaf fall and before bud break. However, the UK’s maritime climate means that ground conditions are often not suitable and most commercial harvests are carried out in the spring, summer or autumn.
Harvesting is the single largest cost component of willow biomass production accounting for about 32 – 60% of costs over the life cycle of the crop (20 plus years).
Several ways of mechanical harvesting have been explored. The direct cut and chip method is most widely used in the UK. In the past, whole stem harvesters and baling systems have been available. Small scale growers also manually harvest with chainsaws or use forest excavators with tree shears.
Efforts to reduce harvesting costs by improving the performance and reliability of the harvesters and chip collection system are essential for the profitability of willow production.
Introduction
Willow is a short rotation woody crop that rapidly produces large amounts of biomass. The harvested willow biomass can be processed into renewable energy, heat, and other products such as livestock and poultry bedding, biochemicals, bio-packaging, and more. Willow is harvested in a three-to-four-year rotation cycle after establishment and can be harvested seven or more times before replanting. It requires minimal crop maintenance between harvests.
Harvesting is the single largest cost component of willow production, accounting for about 32 – 60% of costs over the life cycle of the crop (20 plus years). As such there should be efforts aimed at reducing the harvest cost by having reliable high performing harvest systems to improve the harvesting efficiency and increase the profitability of willow biomass production. One of the Biomass Feedstocks Innovation Programme projects (Net Zero Willow) is aiming to do this by producing a tracked harvester with integrated bunker.
Before planting an SRC plantation, the logistics of harvesting must be considered. For instance, it is important to create an efficient plantation design based on the harvesting method (mechanical or manual) and factor in headlands (unplanted areas around the edge of a crop field) to accommodate the efficient turning of machinery and reduce harvester downtime.
Timing of willow harvest
Willow harvesting should be carried out on a three-to-four-year rotation cycle. Current practice is to harvest three years after the initial planting. Previously, growth in the establishment year was cut back to promote coppicing. However, commercial experience suggested that this led to lots of immature plants being killed before they could properly establish.
Willow harvesting should be done during the dormant season in winter (mid-October to early March) after leaf fall and before bud break in early spring.
The ideal situation would be to harvest willow when the ground is frozen. This avoids excessive loads on the soil by the harvesting machines. However, such optimal conditions are hardly ever feasible in the UK’s maritime climate with mild winters.
However, harvesting windows are not exact. The timing can depend on several factors such as the yield of the crop, the soil conditions and the availability of harvesting contractors. Harvesting can be delayed for a year or two if the growth of the plant is poor due to drought or competition from weeds, insects, or pests. In some circumstances there is no choice but to delay harvesting until the following year especially if weather conditions are unsuitable. Experience suggests that harvesting in poor conditions leads to soil damage and erosion resulting from deep furrows forming in the field. It can also lead to additional costs borne by the contractor due to tyre punctures caused by slipping and sliding into previously harvested stools. Climate change is making the timing of harvests even more difficult.
Most commercial harvesting currently takes place in spring, summer or autumn. This is far from ideal as the woodchip will be contaminated with leaves, which increases the moisture and ash content and makes the biomass fuel more abrasive to boiler systems. Furthermore, the harvested plant biomass removed from the field deprives the soil of carbon transfer and nutrients recycled into the soil.
Harvesting Systems
There are various ways of harvesting willow involving either a single step or two-step method of harvesting. With the single step, the willow crop is cut directly from the stump and chipped in one operation. While with the two-step harvesting, the willow crop is first cut, stored, and naturally air-dried before later chipping and processing into the desired end product. In most situations mechanical harvesting is the only option. In small plantations (usually less than 3 hectares) it is logistically possible to manually harvest and remain economically viable.
Mechanical Harvesting
Mechanical harvesting is the only option for harvesting largescale willow plantations, where the large capacity of the machines can be fully utilized, and the high capital cost can be spread over large harvest volumes. Studies have shown that to increase profitability, it is essential to have machinery available that can produce high-quality wood chips at low cost and be easily managed during storage and transport. There are five contractors currently operating in the UK. They operate various modified forage harvesters that cut and chip willow in a single pass.
Cut and chip harvest system
With the cut and chip harvester, the willow plants are harvested in a single operation and directly chipped and transported to the end user or storage site. The harvester either pulls its own trailer to collect the harvested material or more usually uses a tractor-trailer combination, which travels alongside the harvester and receives the chips blown from the harvester. To optimise efficiency, the harvester downtime should be reduced by keeping the harvester moving and harvesting the willow crop on a continuing basis, while two or three tractors and trailers work in tandem to continuously collect the chips and move them to the load staging area or directly to the end user. The plantation design and staging area should be well laid out to facilitate machinery movement and turning at the end of the rows. Chips produced from the cut and chip harvester, however, have a high moisture content and may require further drying to reach lower moisture content for efficient thermal combustion into energy. Dry chips can attract higher prices from the consumer but this is generally offset by the requirement for additional handling, storage, and fuel costs required for direct drying.
Manual Harvesting
Manual harvesting involves the felling of the willow plants with a chainsaw or brush cutter and collecting the logs manually or with a tractor, followed by direct feeding of the logs into a chipper. Alternatively, the logs can be collected and stored and chipped later. Manual harvesting is labour intensive, requiring a minimum of two persons, and is best suited for harvesting small scale plantations, but is less costly and therefore an affordable option for small scale farmers who lack the resources to purchase expensive harvesting machinery. It can also be used in situations where it is the sole available option when commercial operated machinery is not available when needed, although it is less efficient and less productive compared to the use of mechanical harvesters (one report indicated that it takes on average 45 hours to manually harvest 1 hectare of willow containing about 18,000 plants). A study reported harvesting cost varying from €16.3 ha−1 to €23.2 ha−1, suggesting manual harvesting an affordable option for small scale farmers even though gross production rates are very low (0.10 – 0.11ha/h to cut and lay). Another study showed that manual harvesting exposes workers to noise, uncomfortable work postures, and high cardiovascular loads. This study suggested that motor manual harvesting operations should consider the compatibility of equipment and operational conditions to the workers undertaking such tasks.
It should be noted that manual harvesting results in the stool height rising as chainsaw operators do not always go to the base of the stems. This makes it almost impossible to revert back to the direct cut and chip method in the future.
Latest Technical Articles
Eucalyptus as a Biomass Crop
Key messages:
- Eucalyptus species display rapid growth characteristics, high water and nutrient-use efficiency, and have been identified as having great potential for use in short rotation forestry (SRF) or coppice (SRC) in the UK.
- Low temperatures and unseasonal temperature fluxes are the main limiting factor to Eucalyptus growth.
- Eucalyptus species and provenance must be selected and matched appropriately on a site-by-site basis.
Introduction
The Eucalyptus genus is native to Australia where species have adapted to grow under a wide range of climates and challenging environmental conditions. A number of Eucalyptus species have been identified as having great potential as a short rotation forestry (SRF) or coppice (SRC) species in the UK. Eucalyptus is suited to short rotation forestry due to its fast growth rate and high biomass yield, which can exceed that of other native species. When planted in favourable locations growth rate can be 2 – 3 m per year and can be harvested from 4-6 years onward. Based on an 8-10 year rotation, data from early UK trials identified yields in the range of 20-30 m3 ha/yr, on a bark-free basis, equivalent to 10-15 oven dry tonnes per hectare per year. Yields of up to 48 m3 ha/yr have been reported from a commercial plantation in Cornwall. Eucalyptus produces a high-density wood, with a net calorific value of 18 GJ t, which seasons quickly and is suitable for use as a biomass fuel or timber product.
Site Suitability
Low temperatures and unseasonal temperature fluxes are the main limiting factor to Eucalyptus growth. Frost damage can be a problem during establishment, but risk can be mitigated by proper species selection and silvicultural management.
In general, there is an inverse relationship between frost hardiness and growth rate. E. nitens, for example, displays exceptionally fast growth rate but is more susceptible to frost, this restricts its growth-range to southern Britain. Species such as E. glaucescens and E. gunnii are more cold- tolerant. Mountain-type E. coccifera and E. urnigera have been shown to perform well in exposed conditions and have been established successfully as far north as Moray in Scotland. Other species such as E. rodwayi are swamp varieties which are suited to wetter regions and ground conditions.
Species | Growth rate | Frost hardiness |
E. nitens | **** | * |
E. denticulata | **** | ** |
E. globulus ssp. Bicostata | **** | * |
E. glaucescens | *** | *** |
E. johnstonii | *** | ** |
E. delegatensis | *** | * |
E. regnans | *** | * |
E. gunnii | ** | *** |
E. coccifera | ** | ** |
E. dalrympleana | ** | ** |
E. urnigera | ** | ** |
E. rodwayi | ** | ** |
A table listing some of the main species suitable for use in the UK, including indications of growth rate and frost hardiness is included below; adapted from a review of Eucalyptus in the British Isles.
Eucalyptus species and provenance must be selected and matched appropriately on a site-by site basis. Factors of water availability, elevation, likelihood of unseasonal frosts, soil type and light levels must all be considered.
Establishment
Ground can be prepared via traditional cultivation or planting using a no-till approach. Physical or chemical weed suppression is required post-planting. Alternatively, planting can also be performed through a geotextile membrane to suppress weeds.
Planting is performed from seedlings at stocking densities of between 1,600-2,500 stems per hectare at (2.5 x 2.5m – 2.0 x 2.0 m spacing). 2-3 ha is considered the minimum area for viable commercial production. Tree-shelters and stakes are generally required to protect young plants and support early growth. Due to rapid growth rates, close monitoring is essential for the first few years until trees successfully establish.
- E. dalrympleana, Devon, aged 14 months.
- E. denticulate, Devon, Aged 14 months.
The primary objective is to achieve canopy closure as soon as possible and create a suitable microclimate for trees to thrive. Mixed species stands, or planting with other tree species in shelterbelts, can improve resilience and offset risk. For example, more cold tolerant species can be included on exposed stand edges. Mixed-age stands can also reduce risk from frost damage and exposure by providing shelter for younger trees by providing a positive microclimate and protected environment. Staggered planting and harvesting can be performed to provide continuous self-supply or be adapted to suit farmland available to fit around core farm business and provide other benefits.
Eucalyptus light canopy can afford options for growth in the understory. This means stands are well suited to agroforestry use (silvo-arable/pastural) as well as in shelterbelts, riparian buffer strips and sight screens.
Management, pests and diseases
Leaf loss is a common sign of stress in Eucalyptus trees and should be watched out for. Few pests are currently prevalent in UK. Psyllids are a common infestation on establishing trees but generally have minimal impact. The leaf beetle, Paropsisterna selmani has posed problems in Ireland and eucalyptus gall wasp (Ophelimus maskelli) could pose a threat in the UK. Eucalypts have displayed good resistance to Phytophthora and other foliar pathogens. Young growth can be palatable to deer but the oils in the foliage deter extensive browsing of the foliage. Fencing may be required in areas where these animals are prevalent.
Harvesting
Harvesting is performed using conventional forestry methods. First thinning can be done from year 4-6. At higher stocking densities, and under suitable growing conditions, around 16 t ha can be expected at 1st thinning. Commercial data suggests an annual sustainable yield of around 40m3 ha yr can be achieved, producing consistent roundwood for chipping or firewood use from year 4-5 onward.
- Four-year-old E. nitens stand grown in Cornwall. Trees were 12m in height, 12cm average diameter (dbh)
- Logs produced from first thinning (year 4)
Coppicing – most Eucalypts will coppice well and their capability to do so also mitigates risk if damage to the main stem occurs due to frost or browsing. Coppicing can be performed from years 2–7 and stools will remain productive for 3-4 rotations of 4-8 years, after which the stool viability will drop.
If chipping wood: harvest time, storage conditions and moisture content of the chip needs careful consideration. Higher moisture content can lead to increased fungal growth (composting) in chip stack and under some conditions can also present a fire risk which needs to be accounted for in management of the chip.
Eucalyptus wood can retain higher concentrations of chlorine in the biomass which can cause corrosion in boiler systems if significant quantities are burned. However, this risk can be offset by biomass mixing or pre-treatment. There is also evidence chlorine content decreases with stand age.
Additional uses and benefits
- Eucalyptus foliage is widely used in floristry.
- Eucalyptus attracts pollinators.
- Eucalyptus honey production
- Volatile oils from foliage have potential for multiple applications in nutraceutical and pharmaceutical products such as antiseptic and anti-inflammatory products.
Summary of promising Eucalyptus species for SRF in the UK
|
|
|
|
Further information:
Forestry Commission – Grant funding for woodland
Forest Research – Wood fuel supply guidance
This article was produced in collaboration with Brian Elliott of Eucalyptus Renewables Ltd.
Watch Bryan Elliott’s Biomass connect webinar: Eucalyptus as a short-rotation forestry crop for the UK
Latest Technical Articles
Biomass Buffer Strips – using biomass crops in multipurpose land management
Key messages:
- Buffer strips provide an effective land management option to reduce flood risk, soil erosion, and groundwater pollution from agricultural land.
- Buffer strips consist of planting strips of either grasses, other herbaceous perennials or tree species in 6-20 m wide strips along field margins or beside watercourses.
- Perennial biomass crops such as energy grasses (e.g. miscanthus, switchgrass, reed canary grass) and short-rotation trees (e.g. willow coppice and poplar) can all be used to create buffer strips. Their suitability needs to be matched to the buffer functions required, the site conditions, and planting location.
- For harvesting biomass, site logistics such as access and operating space for harvesting machinery needs to be considered in planning the locations of biomass buffer strips.
Introduction
A buffer strip is an area of land which is either left uncultivated or planted with perennial grasses, shrubs and trees. Planting strips or alleys of perennial biomass crops on existing agricultural land can provide a number of important benefits in terms of flood management, soil recovery and improvements in biodiversity, in addition to providing a harvestable resource. Wider adoption could help better manage landscapes to be more resilient to the effects of climate change, mitigate flood risk, and reduce the environmental impacts of intensive agriculture.
How are buffer strips used?
Buffer strips have been proven to be effective at reducing soil erosion, leaching and run-off of agricultural chemicals which is known to result in pollution of watercourses.
Perennial buffer strips planted adjacent to arable land have been shown to reduce soil sediment, nutrient loss, and pesticide drift while improving soil health and farmland biodiversity. Agroforestry systems have also been shown to provide effective windbreaks, provide wildlife corridors that can connect fragmented habitats and can also enhance the natural regulation of pests. Provided the biomass crops can be safely and effectively harvested, their inclusion in buffer zones adds value by providing an additional harvestable resource which requires little or no maintenance. The biomass crops can make use of nutrient run-off, providing higher biomass yields while simultaneously reducing the nutrient load entering water courses which is a major source of pollution.
Broadly speaking, based on their location, buffer strips fall into one of two categories:
- In-field strips – across the slope of the field, along land contours and field margins.
- Riparian strips – flanking the banks of watercourses.
- Buffer Strips
- Riparian Buffer Zones
The current Environmental Land Management (ELM) scheme in England supports the adoption of buffer strips and there are woodland grants and incentives available for establishing and maintaining woodland for nature recovery, riparian buffers, improving water quality and reducing flood risk but biomass crops and short-rotation forestry (<8 yr rotation) are not currently eligible under current rules.
Use of buffer strips was piloted under the Catchment Sensitive Farming partnership, co-ordinated by Natural England and the Environment Agency, 3836 riparian buffer strips were established on participating farms in England between 2006-2018 and proved a popular measure, with 1 in 6 farms adopting their use. In-field and riparian buffer strips were both within the top-five most impactful catchment-sensitive farming measures identified. Use of biomass crops in buffer strips has great potential, but their adoption has so far been limited.
The main functions of buffer strips.
The main functions of buffer strips can be summarised as: Natural flood management, nutrient and pollution control, protection of soils and nature conservation.
Natural flood management – Buffer strips containing tall grasses, trees or shrubs act as a physical barrier which slows the run-off of surface water following heavy rains making them effective natural flood management strategies which are likely to be of growing importance. Buffer strips can help achieve this by acting in the following ways:
- Increasing the interception of rainfall – leaves catch rain before it reaches the ground slowing the rate of flow and allowing a proportion of the rainfall to evaporate from the leaf surface.
- Slowing the flow of surface water – above-ground vegetation provides a physical barrier which slows the flow of water running off the soil surface.
- Increasing absorption of water into the soil – Established buffer strips can improve soil structure allowing water to penetrate deeper into the soil, increasing the volume that can be stored in the soil and reduce the likelihood of saturation which leads to greater run-off.
- Larger perennial crops and tree species take up significant quantities of water from the soil, can help reduce soil saturation and ameliorate areas prone to waterlogging. Conversely, in drier conditions, buffers can also help to conserve soil moisture and reduce effects of drought.
Pollution control – In areas where agricultural land is more intensively managed, nitrate-N (NO3–) and phosphorous (P) leaching and run-off is known to be a main source of pollution resulting in eutrophication of lakes and waterways. Deep rooting perennial plants and tree species can act as a biological filter which take up and utilise nutrients before they enter watercourses. Studies have shown that riparian buffers can remove between 30-99% of nitrate and 20-100% of phosphorous depending on site conditions and the buffer composition, with combinations of grasses and trees tending to be most effective. In situations where frequent incidences of fertiliser run-off are common, some riparian buffers have been shown to reach saturation after a few years if the biomass is not regularly removed. For this reason, using biomass crops in buffers provides an added benefit as the leaching fertiliser can result in higher harvestable yields of biomass and also help maintain the effectiveness of the buffer strip in terms of N and P uptake. In addition to nutrient run-off, buffer strips can also be effective for wastewater management. Biomass crops such as willow short-rotation coppice have been shown to be particularly effective, permanent nutrient removal of 55 kg PO43−eq ha yr has been reported based on field trials conducted in Northern Ireland.
Pesticides are another potential source of pollution from agricultural practice. The pesticides applied in the UK have a wide range of different properties and modes of action, but in general most pesticides will preferentially adsorb to dead organic material such as leaf litter. Incorporation of tall, dense and fast-growing species in the buffer strip can also act as a windbreak or shelter belt which can create a physical barrier to prevent pesticide drift. Biomass crops which create deep leaf litter layers before the biomass is harvested, such as miscanthus and willow, may also be particularly effective if protection from fertiliser run-off and pesticide drift are both required. Poplar and willow have been shown to take up and immobilise a wide range of pollutants, including pesticides.
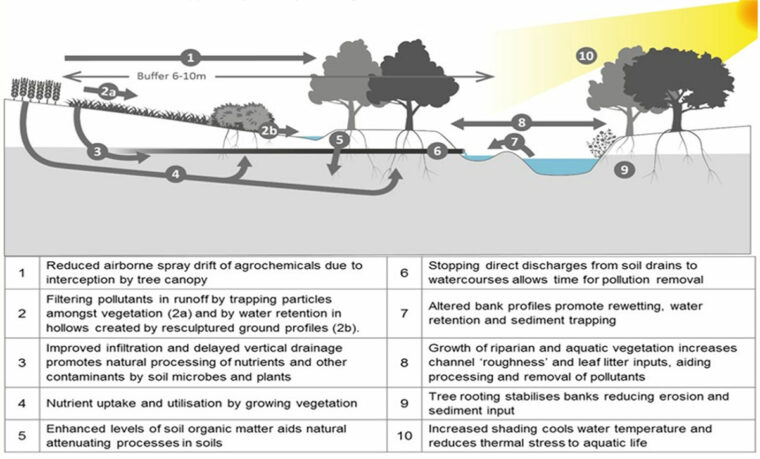
Source: Environment Agency (2020). 3D buffer strips: Designed to deliver more for the environment. Environment Agency, Bristol.
Soil health – The above ground vegetation of the buffer strip helps catch and retain soil that has been eroded by wind or water. On degraded arable soils, uncultivated buffer strips will also allow the soil to recover over time. Deep rooting plants can help stabilise soils at higher risk of erosion, such as riverbanks and areas around water bodies, often collectively referred to as riparian zones.
Buffer strips have also been shown to enhance soil carbon through sequestration, root turnover, leaf litter, and simply by leaving the area unploughed for a prolonged period which allows the soil time to recover and reduces incidences of soil compaction. The total carbon stocks under short rotation forestry have been reported as 1.5 times higher than alternative land uses when planted on agricultural land.
Nature conservation – Biodiversity and wildlife corridors – Buffer strips provide important habitats and can provide cover for wildlife to allow them to move freely between different habitats which are often fragmented by field boundaries. Tall grass and tree species incorporated into buffer strips also provide shade which can be of benefit to aquatic life in water courses. Perennial biomass crops also provide important winter cover. As they are harvested in late winter, they provide vegetative cover when adjacent agricultural land is often bare which also poses even greater risk of flooding and erosion.
Perennial biomass crops best suited to use in buffer strips
A number of different biomass crops may be suitable for use in buffer strips. Perennial grasses bred specifically for high-yield and energy content are one option. Stiff-stemmed grassland species that are resistant to high run-off rates are most suitable and can be selected as a feedstock for multiple purposes: Combustion, anaerobic digestion, forage/grazing or animal bedding. Wildflower mixtures are also an option for inclusion. Some energy grasses, such as miscanthus, may require a minimum planting area and an established local market in order to be economically viable to harvest on smaller scales, unless used on-farm as animal bedding.
Short-rotation coppice (SRC), e.g. tree species such as willow and poplar, which are cut to a low stump (stool) and produce multiple stems that are harvested every 2-5 years, are also an option where land is not too steep. Alternatively, short-rotation forestry (SRF) may be suitable. SRF uses high-yielding tree species grown at high stocking densities for 8-20 year rotations. Wood is harvested when stems reach approximately 20cm diameter. If space permits, conventional woodland management of wooded buffer zones is a long-term option.
Types of buffer strip
In terms of composition, buffers can be classed as: Vegetated, incorporating grasses and wildflower species; Wooded, incorporating tree and shrub species; or integrated/engineered, incorporating a mixture of species and/or earthworks. Integrated or engineered buffer strips are recommended as the best designs for incorporating biomass crop species. With reference to riparian buffer strips, a three-zone structure has been recommended as most effective for use with biomass crops:
- Grass strip – This strip reduces the flow rate of surface run-off, filters sediment and reduces erosion. Nutrients are taken up by the plants during the growing season. The strip may be composed of any perennial grasses, including energy grasses. Depending on the grass used, the strip can also be used for extensive grazing, silage/hay production, animal bedding, or for bioenergy.
- Woodland strip – This strip improves interception of rainfall, deep rooting tree species help stabilise banks and filter nutrient and pollutant run-off via uptake and immobilisation in the biomass. Taller species also act as windbreak and prevent erosion and pesticide drift. This strip can be composed of mixtures of short-rotation coppice (SRC) or short-rotation forestry species (SRF). The harvested biomass can be chipped or cut as billets for firewood.
- Undisturbed strip – helps to protect planted strips during flooding events, reduces bank erosion and provides a buffer between planted biomass crops and water courses to allow space for harvesting and maintenance operations.
Slope can be used as one of the key considerations for planning a biomass buffer
Low gradient |
|
Intermediate gradient
|
|
Steep Gradient
|
|
Other considerations
Depending on where buffers are located, existing land drains can negate the effects of buffer strips due to providing a bypass for surface water run-off. In cases where extensive networks of land drains are already present, redirecting flow to a drainage ditch between the field and the buffer could provide a solution. Where this is not possible, a constructed wetland area could be another option for environmental protection and conservation.
The greater the level of engineering that goes into buffer strips, the greater the cost, but also the greater the benefit. Planning needs to take account of biomass crop establishment and harvesting requirements both in terms of cost and management considerations.
Where livestock are also present, or in areas where browsing damage from rabbits/deer is a problem, fencing may be required to prevent grazing of establishing buffer strips and to keep livestock away from water courses.
Biomass Connect Media:
Dr Chris Johnston of the Agri-Food and Biosciences Institute (AFBI), a Biomass Connect partner, gave talk at the UK Low Carbon Agriculture Show, February 2023, showing results of field trials into the effectiveness of short-rotation coppice willow for environmental protection and wastewater management.
Current UK policy
The current Environmental Land Management (ELM) scheme in England supports the adoption of buffer strips and there are woodland grants and incentives available for establishing and maintaining woodland for nature recovery, riparian buffers, improving water quality and reducing flood risk. Biomass crops and short-rotation forestry, with rotation length less than 8 years, are not yet eligible for ELMs or woodland creation (EWCO) incentives.
Conclusion – Main considerations when establishing biomass buffer strips.
The optimum design, composition and width of a buffer strip depends on a wide range of factors which include:
- The environmental and conservation issues being addressed.
- The previous and adjacent land use.
- The soil type and condition.
- The slope, topography and hydrology of the site.
- The desired biomass products and yields.
- Harvesting technology required and local markets available for the biomass.
- Cost of measures required and government payments available to support their use.
Further information:
Related Biomass Connect Articles:
How does Short Rotation Coppice (SRC) willow affect biodiversity?
What effect does planting biomass crops have on soil carbon?
Other resources:
Funding for landowners – Nature based solutions to reduce flood risk
Examples and case studies – How nature-based solutions can mitigate flood risk.
Riparian forest management on freshwater environment – Best management practice guide.
Natural Flood Management Handbook – A practical guide for farmers.
Latest Technical Articles
Biomass Pelletisation: Influence of biomass characteristics on pellet quality
Take home messages:
- Biomass pelletisation is the process of condensing biomass material into energy-dense pellets by forcing the biomass material under high pressure and temperature through a pellet mill to produce small cylindrical pellets.
- Biomass pellets have uniform size and shape, high bulk and energy density, and low moisture content, all contributing positively to the supply chain management and logistics cost.
- The quality and characteristics of biomass pellets are determined based on key parameters such as the type of biomass feedstock, moisture content, particle size, binding material, pressure, and temperature.
- A high-quality pellet should be dry, hard, durable, and with low ash content. The ENplus certification scheme outlines pellet properties and related threshold values.
Introduction
The global demand for biomass is increasing in response to the need to reduce dependency on fossil fuels, concerns about environmental problems related to its use, and the increasing desire to mitigate climate change. Biomass raw materials are widely regarded as a significant renewable energy source and an alternative to fossil fuels. However, there are limitations with the use of biomass feedstock in its natural form for energy conversion systems. Biomass feedstocks are bulky, have low bulk density, non-uniform properties, low energy density, and high storage, handling, and transport costs. One way to overcome the limitations is using densification to convert biomass feedstock into pellets before use for energy purposes.
Biomass pellets are biomass materials compressed into high energy dense pellets, characterised by; homogenous shape and size, low moisture content, higher bulk density, thus reducing the storage, handling and transportation cost and lower environmental impacts. Biomass pellets could be used directly as fuel for residential heating stoves, heating boilers and large-scale power plants. Biomass use in the form of pellets generates a biofuel that is more cost-effective than the direct use of non-modified biomass residues for energy production. This article explores the critical issues in the biomass pelletisation process, biomass pellets characteristics and standardisation of high-quality biomass pellets for energy production.
Pellet production process
Biomass pelletisation is the process of condensing biomass material into energy-dense pellets by forcing the biomass material under high pressure and temperature through a pellet mill consisting of a die with cylindrical press channels and rollers, to produce small cylindrical pellets. The friction between the biomass and the press channel generates a force compressing the biomass into pellets that are dense and cut at a uniform size. The biomass pelletisation process consists of multiple steps including pre-treatment (particle size reduction, drying, and conditioning), pelletising, and post-treatment (cooling, screening, packaging).
Pre-treatment involves reducing the biomass particle size and drying to reduce the moisture content. The harvested biomass is processed into chips and fed into a mill, further reducing the particle size in order to avoid blockage in the pellet mill. Studies have found that biomass materials with small particle sizes and a large surface area during pelletisation result in a higher density, and stronger pellets.
Following the particle size reduction, the biomass material goes through a drying phase where the material is dried in a dryer. The moisture content affects the overall quality of the final pellet produced. Depending on the biomass material and the lignin content, additives or binding agents may be added to improve the quality of the final product. Studies have shown the need to incorporate additives to biomass feedstock when pelleting biomass materials with zero or low lignin content. Lignin is one of three major components of lignocellulosic biomass with binding attributes. Additives such as starch, corn flour, potato flour, vegetable oils, etc., which are materials intentionally added into the pellet production or added after production should not exceed two percent of the total pellet biomass. Additives have the benefit of improving the quality of pellets, reducing emissions, increasing production efficiency, or marking the pellets.
The pelletisation process takes place in a pellet mill. The pellet mill has basic components of a die and cylindrical press channel and rollers. The friction between the biomass and the press channels causes pressure to build and increases the temperature of the biomass material which softens the lignin content, binding the biomass material and compressing the biomass into small cylindrical pellets.
After pelletisation, the biomass pellets are allowed to cool to solidify into more durable forms. Once cooled, the biomass pellets are stored or packaged, ready for use. The fine materials that are not formed into pellets are recycled back into the pelletisation process.
Pellet quality requirement
Pellets produced from biomass should meet international pellet standards. The UK Pellet Council (UKPC) manages the license for ENplus pellet quality certification in the UK, ensuring adherence to the standards and the production and delivery of high-quality wood pellets for heating. The ENplus certification scheme defines three pellet quality classes (ENplus A1, A2 & B) which are based on the ISO 17225-2. The ENplus certification scheme outlines pellet properties and related threshold values.
Table 1: Pellet quality requirement | ||||
Property | Unit | ENplusA1 | ENplus A2 | ENplus B |
Diameter | mm | 6-8 | ||
Length | mm | 3.15<L≤40 | ||
Moisture content | % a.r | ≤10 | ||
Ash content | % a.r | ≤0.7 | ≤1.2 | ≤2.0 |
Mechanical durability | % a.r | ≥98.0 | ≥97.5 | |
Dust/Fines (<3.15mm) | % a.r | ≤0.7 | ||
Net calorific value | MJkg-1 a.r | ≥16.5 | ||
Bulk density | Kgm-3 | ≥600 | ||
Additives | % a.r | ≤2.0 | ||
Nitrogen | %d.b | ≤0.3 | ≤0.5 | ≤1.0 |
Sulphur | %d.b | ≤0.04 | ≤0.05 | |
Chlorine | %d.b | ≤0.02 | ≤0.03 | |
Ash deformation temperature | oC | ≥1200 | ≥1100 | |
Arsenic | Mg/kg d.b | ≤1 | ≤1 | ≤1 |
Cadmium | Mg/kg d.b | ≤0.5 | ≤0.5 | ≤0.5 |
Chromium, Copper, Lead, Nickel | Mg/kg d.b | ≤10 | ≤10 | ≤10 |
Mercury | Mg/kg d.b | ≤0.1 | ≤0.1 | ≤0.1 |
Zinc | Mg/kg d.b | ≤100 | ≤100 | ≤100 |
Symbols refer to a.r= as received, d.b= dry basis | ||||
ENplus Handbook, version 3.0, part 3. |
Biomass feedstock has an influence on the quality of the pellets produced. A high-quality pellet should be dry, hard, durable, and with a low ash content of less than two percent. According to the ENplus standards, the length of the pellet varies ranging from 3-40mm and a diameter of 6-8mm. Biomass pellets should have a moisture content of less than 10 percent. Dust produced during the processing stages of biomass collection, size reduction and pelletisation should be minimal (no more than 1 percent). Dust is highly combustible, leading to explosions and imposing health hazards.
Furthermore, quality pellets should be durable with high bulk density. Particle bonding by the introduction of additives is considered a vital event in biomass pelleting. The production of durable pellets is a function of the lasting attraction between individual particles during biomass pelleting. Where additives are added into the pellet production or added after production, it should not exceed two percent of the total pellet biomass.
Parameters affecting the quality of produced biomass pellets
The quality and characteristics of biomass pellets are determined based on key parameters such as the type of biomass feedstock, moisture content, particle size, binding material, pressure, and temperature.
The type of biomass feedstock influences the quality of the pellet. Different feedstocks have different characteristics and energy requirements for pelletisation, which directly impacts production cost and production capabilities. Biomass materials often used for pelleting are wood from forestry, followed by agricultural residues. The limited availability of wood resources and increasing global demand for biomass pellets has resulted in efforts to broaden the raw material base used for pellet production, including short rotation coppice biomass crops and herbaceous perennial biomass such as Poplar (Poplar spp.), Miscanthus (Miscanthus giganteus), and switch grass (Panicum vigatum). For instance, a study found poplar as a suitable material for producing pellets that meet many of the pellet quality standards (see Table 1) required on the market. Studies on Miscanthus pelletisation have however often found the use of Miscanthus biomass producing pellets of low durability and high ash content and requiring high energy inputs. The lower lignin content and poor bonding between particles have been identified to account for the low pellet quality. The recommendation to improve the pellet quality of biomass with such characteristics is the addition of binders and mixtures of different raw materials as they affect the quality of the pellets.
Biomass feedstock with optimal moisture content is essential for producing quality pellets with better mechanical durability, strength, and thermal conversion performance. Recommended moisture content ranges from 10-15%. Moisture content affects the quality of pellets especially if pellets are to be stored before use. Research has shown that decreasing moisture content will increase the density and durability of pellets. Increasing the moisture content above the recommended optimum values however, has negative influence on the pellets mechanical durability and reduces the pellets density.
The applied pressure during the pelletisation process affects the natural binders such as lignin, starch, protein, and water-soluble carbohydrates present in the biomass material. As pressure increases up to a certain point, these binding components are pressed out causing the biomass particles to bond together. Studies have shown a positive effect of increasing pressure on various biomass feedstocks quality by increasing the durability and hardness of the produced pellets. Also, research has shown optimal die temperature for pelletising biomass feedstock is close to 100 °C.
Furthermore, the particle size of the biomass feedstock depends on the biomass characteristics, particle size reduction methods during pre-treatment and the pelletising equipment. Decreasing the biomass particle size increases friction in the press channel of a pellet mill resulting in increasing pellet density.
Conclusion
The global demand for pellets is increasing in response to the need to reduce dependency on fossil fuels and concerns about environmental problems related to their use. Biomass pellets are produced from woody and non-woody biomass feedstocks through a process of condensing biomass material into energy-dense pellets by forcing the biomass material under high pressure and temperature through a pellet mill to produce small cylindrical pellets. Biomass pellets have uniform size and shape, high bulk and energy density, and low moisture content, all contributing positively to the supply chain management and logistics cost. The quality and characteristics of biomass pellets are determined by key parameters such as the type of biomass feedstock, moisture content, particle size, binding material, pressure, and temperature. A high-quality pellet should be dry, hard, durable, and with low ash content. The ENplus certification scheme outlines pellet properties and related threshold values.
Latest Technical Articles
Bioenergy with Carbon Capture and Storage (BECCS)
Key messages:
- Bioenergy with carbon capture and storage (BECCS) involves generating heat and power from biomass fuels, capturing the emissions released and storing them.
- BECCS represents one of only a few technological options currently available to remove historic CO2 emissions from the atmosphere.
- The UK government has stated that future large-scale biomass power facilities will not be supported without the inclusion of CCS.
- To achieve net-negative emissions reduction via BECCS, significant quantities of land will be required to grow dedicated energy crops. This must be managed sustainably to prevent negative impacts on biodiversity and competition with food production.
Introduction
Bioenergy from plant biomass coupled with carbon capture and storage (BECCS), is regarded by the UK government as one of the key greenhouse gas removal (GGR) mechanisms currently available to avoid global warming passing beyond 1.5°C. BECCS involves using plant biomass to remove historic CO2 emissions from the atmosphere, generate power, and subsequently capture the carbon emissions released and store them long-term. The UK Climate Change Committee have estimated BECCS could sequester between 20 – 65 Mt CO2 equivalent per year by 2050, equivalent to 15% of the current UK CO2 emissions.
What are Carbon Capture and Storage technologies?
The overall purpose of carbon capture utilisation and storage (CCUS) is to stop carbon dioxide (CO2) emissions from combustion reaching the atmosphere and adding to global warming. Instead, the CO2 produced is captured at source and is either utilised in another industrial process (CCU) or stored deep underground in geological formations (CCS).
The British geological survey has described CCS as ‘the oil extraction industry in reverse’: replacing natural gas and oil removed from the earth with CO2 produced from the combustion of it. The process is already in use in existing oil recovery operations where it used by oil companies to extract more oil from existing wells.
While use of CCUS to capture CO2 released from the burning of fossil fuels would undoubtedly help reduce future emissions, it does nothing to help reduce the levels of CO2 that have already been released into the atmosphere and already driving climate change. While other ‘direct-air’ carbon capture (DAC) technologies are also being developed, BECCS has the potential to be deployed more swiftly because it utilises the existing biology of plants to capture historic CO2 from the atmosphere and store it. The technology this requires can be retrofitted to many existing facilities. This provides options for decarbonising industries that are already emitting significant quantities of CO2, such as power stations, cement production and steel manufacture. If sufficient plant material can be grown, harvested and transported sustainably, BECCS could offer a viable means of reducing global carbon emissions.
How does BECCS work?
While plants are actively growing, they are removing CO2 from the atmosphere and storing it in their bodies – essentially nature’s own version of carbon capture and storage. Plants capture CO2 from the atmosphere during photosynthesis; where CO2 combined with energy from the sun, water and nutrients from the soil, is converted into biomass that is used to build their above and below-ground structures. When the plant dies and rots or is burned, most of the CO2 captured during its lifetime is released back into the atmosphere. However, if the carbon emitted from burning biomass is captured and stored long-term, the process potentially becomes ‘carbon-negative’. Put simply, the BECCS process involves carbon being actively removed from the atmosphere by plants to produce a biomass fuel, that fuel is used to generate heat and power, and the emissions produced in the process are captured and stored.
How is the carbon captured?
There are three main methods of carbon capture:
- Post-combustion – Capturing GHGs as they exit the flue after combustion.
- Oxyfuel combustion – Combustion of fuel in an excess of oxygen so it is completely converted to a mixture of CO2 and steam.
- Pre-combustion – Converting fuel to a hydrocarbon gas (gasification) and removing CO2 formed during the process prior to combustion.
Post-combustion and Oxyfuel combustion are most applicable for use by existing power and heat generators as it is possible to retrofit these systems. Pre-combustion processes are more applicable to gasification processes which can be used for heat and power but also for generation of liquid transport or aviation fuels. In this instance the process captures CO2 released during the gasification step of the process, but CO2 will still be released when the hydrocarbon fuel produced is subsequently burned.
The gasses that are captured during this process undergo a process called ‘scrubbing’ where they are passed/bubbled through a solvent media which causes particulates in the gas stream to be removed and the pure CO2 is separated, pressurised to a liquid state, and can then be bottled, piped or transported in the same way as other commercially used gasses. Once in a clean, transportable state the CO2 can either be:
- Utilised (CCU) for other industrial processes: manufacturing, generation of hydrogen or use in plant or aquaculture growth chambers.
- Stored (CCS) by pumping the CO2 into suitable geological formations (>1km) deep below the land or sea.
A comprehensive review of the BECCS processes has been written by Shahbaz et al. (2021).
Where is BECCS currently being used?
Carbon Capture Utilisation and Storage (CCUS) technologies are already being developed in many parts of the world. According to a recent report by the International Energy Agency (IEA), in September 2022, there were 35 commercial facilities in operation collectively capturing approximately 45 Mt CO2 globally, with many more either planned or under development. CCUS is also one of the main elements of the UK governments Clean Growth industrial strategy. According to the IEA, globally over 2 Mt CO2 are currently being captured annually from biogenic (biomass derived) sources, half of which was geologically stored.
- BECCS coupled with bioethanol production currently represents 90% of operational carbon capture facilities. The Decatur Industrial CCS project in Illinois, USA is one of the largest CCS facilities in operation since 2018. The Illinois project captures CO2 from bioethanol production and geologically stores 1 Mt CO2 per year from the process. Another 40 bioethanol facilities are planned, the majority based in the USA, which are estimated to collectively capture 15 Mt of biogenic CO2 by 2030.
- BECCS coupled with heat and power facilities are also under development with 15 Mt planned predominantly from dedicated biomass power facilities and a third from waste-to-energy facilities. In 2020 a large-scale solid biomass plant with CCS was commissioned in Japan but is still identifying suitable geological storage. Europe’s first BECCS Pilot facilities are currently being trialled and developed at Drax power station in Yorkshire, with larger-scale deployment of the technology expected to be operational by 2027.
- BECCS coupled with other industrial processes are also in development including five cement plants in Europe, several pulp and paper production facilities and two hydrogen generation facilities.
CCUS is considered a key technology in the UK governments Clean Growth industrial strategy and the first operational CCUS facilities are planned to be operation within the next five years and deployed at scale over the next decade. The UK governments 2021 Biomass policy statement stipulated that future large-scale biomass power facilities would not be supported without the inclusion of CCS.
The map below shows key UK locations for CCS clusters identified by BEIS (2018) The UK Carbon Capture Usage and Storage deployment pathway: an action plan.
Significant challenges remain
While technical aspects of the CCS process have been in use for some time, much of the infrastructure required for CCS does not yet exist, and there are only a few large-scale demonstrators that have been built or monitored in long term trials. Questions have been raised whether BECCS would be cost effective compared with money spent on other forms of renewables in energy generation, but there are currently few other options that would also help with historic greenhouse gas removal. Concerns have also been raised regarding how safe and secure the carbon dioxide stored below ground would be, how this can be monitored, and how potential leaks could be managed. The EU commission are currently developing a CCS directive to help address these concerns and provide regulation.
The CCC acknowledge that there are other pathways with a better than 50% chance of limiting global warming to below 1.5°C without large-scale deployment of BECCS, but these pathways would require significant and rapid changes in energy use efficiency, shifts in diet, low population growth and large-scale afforestation.
Sourcing biomass sustainably is critical
Global adoption of BECCS will inevitably require large quantities of biomass, and large areas of land will be required to produce it. According to the CCC, land used for energy crops will have a lower carbon content than afforested land, but energy crops produce higher yields of biomass which can be harvested annually (energy grasses) or every 3-5 years (short rotation coppice). For this reason, the CCC have suggested that energy crops used with BECCS can provide greater cumulative long-term carbon sequestration than afforestation provided their supply chain emissions are low. A recent study on use of BECCS in the UK identified that to meet carbon sequestration of 50 Mt CO2 per year by 2050, an estimated 1 million Ha of UK agricultural land would be required, in addition to either importing 6-8 million tonnes of biomass per year or relying on an additional 0.59 or 0.49 million Ha of domestic forest resources.
There are concerns as to whether this biomass can be grown and sourced sustainably without causing other negative impacts on the environment; especially in terms of impacts on ecosystem services, biodiversity, water use and other factors associated with land use change such as trade-offs with food production. The CCC also acknowledge the risk that unsustainable biomass harvesting could pose, both domestically and internationally. Sustainability criteria are applied in the UK to biomass used in heat, power and transport sectors (RED II) in attempt to mitigate negative consequences of biomass use. The EU has begun instituting a law aimed at preventing global deforestation and helping to establish deforestation-free supply chains.
BECCS could provide an important mechanism to reduce emissions and remove greenhouse gasses from the atmosphere. However, full life-cycle assessment of biomass production and supply will be critical to ensure BECCS is truly net-negative and does not result in other deleterious effects on our natural environment or food production systems.